Microbial Genomics: Standing on the Shoulders of Giants
Professor Sir David Hopwood, part II
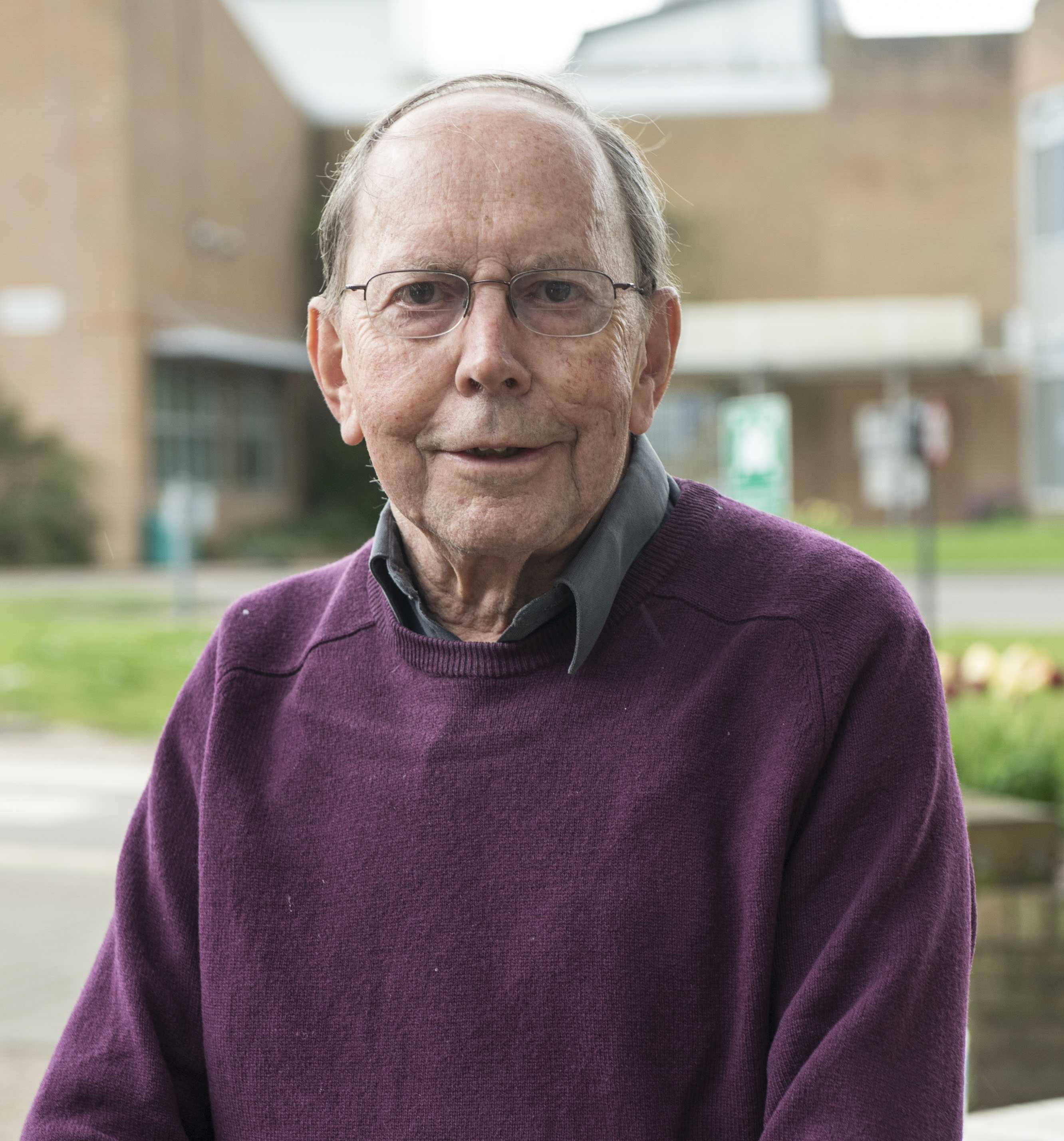
Sixty years of Streptomyces genetics: from Petri dish to computer
From graduating with a degree in botany at Cambridge University, to a PhD in microbial genetics, to co-ordinating the sequencing of the genome of Streptomyces coelicolor, the largest microbial genome to be sequenced at the time, David Hopwood has had a momentous and fascinating career interacting with many great scientists and promoting the development of microbial genomics.
Part II - Artificial recombination by protoplast fusion and gene cloning
In 1976, groups in Szeged, Hungary, and in Paris announced a new way in which bacterial genes – they were working with Bacillus – could be recombined, not by harnessing a natural means of gene exchange like conjugation or transformation, but by an artificial trick involving the fusion of protoplasts. These are cells of plants, fungi or Gram-positive bacteria from which the rigid wall has been removed by treatment with an enzyme – in the case of bacteria this is lysozyme – leaving the underlying plasma membrane exposed to the external medium. The treatment must be made in an osmotically stabilising medium, such as 10% sucrose, to balance the internal pressure inside the cells and prevent the nascent protoplasts from bursting. A few years before, Masanori Okanishi in Tokyo had made a careful study of conditions for making Streptomyces protoplasts and, crucially, for regenerating them back into a normal mycelium (Figure 1), but had not harnessed the protoplasts for genetics. We set out to see if protoplast fusion would lead to genetic recombination in Streptomyces coelicolor.
Helen Wright (later Kieser) prepared protoplasts from two strains, each carrying auxotrophic mutations and lacking the native sex plasmids SCP1 and SCP2 so that if we obtained recombinants they could not have arisen by natural mating. She was about to attempt to fuse them using the conditions described for Bacillus when we had a visit from Guido Pontecorvo, the father of the parasexual cycle in fungi and of human somatic cell genetics. He had been a charismatic giant on whose shoulders I had stood while I was a lecturer in the Genetics Department at Glasgow from 1961 to 1968, after leaving Cambridge and before moving to the John Innes Centre in Norwich. He said, "Use the conditions that work for animal cells – they will be much better than the ones the Bacillus people used." Sure enough, when we took the plates out of the incubator a couple of days later we could hardly believe our eyes. There were nearly as many colonies on the medium selecting recombinants as on those on which parental genotypes grew. Later, when we tested all the variables of medium composition, time of treatment and concentration of the fusing agent, polyethylene glycol (PEG), we never improved on the recombination frequency! We were dealing with an unprecedented situation in bacterial genetics in which complete genomes of two parents came together to give rise to all possible combinations of genes almost as efficiently as in a eukaryotic sexual cycle. We later showed that protoplasts of up to four strains could be mixed together to yield recombinants inheriting genes from all the parents.
Protoplast fusion was a great tool for constructing complex genotypes in academic research but it was also valuable for strain improvement in the antibiotics industry. Dick Baltz at Ely Lilly, who developed protoplast fusion in industrial strains independently of our work, was motivated by the desire to combine yield-enhancing genes from divergent selection lines in classical mutation and screening programmes, as Pontecorvo had first suggested for penicillin-producing fungi in the 1950s, although his words had largely fallen on deaf ears. Later, scientists at Maxygen used our technique of fusing multiple parents, in a recurrent fashion that they dubbed "genome shuffling", to show that the same antibiotic yield could be obtained in one year by screening only 24,000 strains as it had taken Lilly 20 years and the testing of a million cultures in the classical procedure.
The 1970s were heady years for microbial genetics as gene cloning was pioneered at Stanford and UCSF, building on the crucial discovery in Stan Cohen’s group at Stanford of artificial transformation of E. coli, which does not have a natural DNA uptake system. It was persuaded to take up plasmid DNA by manipulating medium and temperature conditions. We wanted to find methods for introducing DNA into S. coelicolor, and it turned out that in Streptomyces protoplasts are the key. But we also needed one or more selectable markers that could assume the role of the antibiotic resistance genes, which were key players in E. coli, to build into a cloning vector. There was no such gene on an isolable Streptomyces plasmid. However, a unique piece of Streptomyces plasmid biology came to the rescue. When a spore of a plasmid-carrying strain germinates on an agar medium surrounded by a large excess of a strain lacking that plasmid, transfer of the plasmid results in a slowing of the development of the recipients, and as the culture develops and the plasmid spreads from the first recipients to their neighbours, the outcome is a visible circle of retarded growth up to several millimetres in diameter that we called a "pock" (Figure 2). Mervyn Bibb found that pocks could be used as a powerful visual indication of plasmid uptake when he added plasmid DNA to protoplasts in the presence of PEG and regenerated them; there was one pock for every 100,000 DNA molecules in the mixture, as high a frequency of transformation as in cloning experiments in E. coli, so evidently we were potentially in business. However, we still needed to build a cloning vector.
Mervyn, who by then had moved to Stanford to work with Stan Cohen, (whom he had got to know while Stan was on sabbatical in my lab in 1976) and my new postdoc, Charles Thompson, aimed to build on a native Streptomyces plasmid, while Keith Chater focused on the phiC31 phage, in homage to the wonderful lambda vectors that had been developed for E. coli. All were successful. Mervyn used, as a selective marker, the resistance gene for methylenomycin that accompanies the biosynthetic genes of S. coelicolor while Charles isolated several self-protecting resistance genes from other antibiotic-producing actinomycete species. Juan Suarez, working with Keith, fused a small E. coli plasmid with a deleted version of phiC31 to give a vector that replicated as a plasmid in E. coli and a phage in S. coelicolor. The way to cloning and analysing Streptomyces genes was open, and an obvious target was antibiotic biosynthesis. Again, the pigmented S. coelicolor antibiotic actinorhodin came into its own.
Paco Malpartida joined me as a postdoc from Madrid in 1983 and we had a wonderful collaboration for the next several years. Using a large-capacity cloning vector, constructed from the SCP2 plasmid, he made a "shotgun" gene library from wild-type S. coelicolor in one of Brian Rudd's actinorhodin mutants (see Part I of this narrative). Amongst some thousands of colourless colonies on the regeneration plates, three were blue so they must have carried the wild-type version of the mutant gene. Restriction mapping showed them to carry overlapping segments of DNA, as expected, and Paco was able to stitch them together to make a clone that complemented all of Brian's mutant classes. Perhaps it carried a cluster of genes for the entire actinorhodin biosynthetic pathway from simple primary metabolites, in this case acetyl and malonyl coenzyme A. Sure enough, when Paco transferred the clone to Streptomyces parvulus, which does not make actinorhodin or any related antibiotic, the recombinant clone turned blue. We must have bagged the complete biosynthetic pathway!
More excitement lay ahead. Actinorhodin belongs to a class of antibiotics called benzoisochromanequinones; let’s call them BIQs. Others include granaticin, worked on by Heinz Floss, then at the University of Ohio at Columbus, and nanaomycin and medermycin, the preserve of Satoshi Ōmura at the Kitasato Institute in Tokyo. They both sent producers of these compounds to Norwich and Helen was able to transfer some of Paco’s actinorhodin clones into them. The most striking result came from experiments with the producer of medermycin, a brown-pigmented compound. Opening the incubator one morning we had a Eureka moment – one of the cultures had turned a beautiful purple colour we had never seen before. It must have been making a "hybrid" antibiotic and chemical analysis in Tokyo confirmed this conclusion (Figure 3). Experiments with the granaticin producer also yielded a novel compound, although in this case there was no colour change so tricky spectroscopy was needed even to demonstrate this. Antibiotic biosynthesis had entered the recombinant DNA age. For me, the most satisfying consequence was a stage in the actinomycete genetics story, in which molecular genetics and natural product chemistry came together to solve fundamental questions about the way these ingenious bacteria make such a mind-boggling array of molecules, many with great medical, veterinary and agricultural utility.
The BIQs are minor players in the natural product field, but they belong to the most important and widespread chemical family, the polyketides, which includes antibiotics such as erythromycin and the tetracyclines, immunosuppressants like cyclosporin and rapamycin, the statins, the antiparasitic agent avermectin, and the anthocyanin pigments. As such, work on the genetics of actinorhodin and its chemical relatives could help in understanding the mysteries of how the polyketides are assembled, stepwise, from simple carboxylic acids such as acetyl, malonyl and methylmalonyl CoA. Chemists and biochemists had realised that this was related to the way in which fatty acids are made, but with much more complex "programming" involving the choice of building units, the reductive status and chirality at each step in carbon chain building and the length of the chain.
David Sherman, a chemist from the Biogen company in Cambridge, Massachusetts, joined my group in 1987 to work on the granaticin gene cluster as a basis for understanding the programming logic, while Mervyn Bibb, who was on sabbatical at the Cetus Corporation in California, collaborated with Dick Hutchinson in Madison on the tetracenomycin cluster. The results from the two studies were the same: the various catalytic functions involved in chain building reside on separate proteins that come together as a non-covalent complex, as in a bacterial fatty acid synthase. Next, David embarked on experiments to see if we could extend the first hybrid antibiotic results, in which the changes involved the action of enzymes at late stages in the biosynthetic pathway – so-called "tailoring" steps – to the early stages of carbon chain building. By mixing and matching genes from the actinorhodin and granaticin clusters, David showed that the subunits could indeed form functional enzyme complexes, even though they had presumably diverged from a common ancestor millions of years ago, auguring well for the future of genetic engineering in antibiotic discovery.
My next postdoc was Chaitan Khosla, a chemical engineer from Caltech, who came to Norwich in 1989 to learn the tricks of Streptomyces genetics and cloning. Pretty soon he was blazing a trail that led, after he returned to California a couple of years later to a faculty position at Stanford, to the concept of "combinatorial biosynthesis of unnatural natural products", beginning to put hybrid antibiotic production on a systematic footing. Chaitan and his postgraduate student, Bob McDaniel, first focused on the aromatic polyketides, including the BIQs, anthracyclines and tetracyclines, and deduced much about the roles of the synthase subunits, notably the determination of carbon chain length, and thus the ability to design novel natural products de novo by combining subunits from different biosynthetic pathways. Meanwhile, Peter Leadlay and his group in Cambridge and Leonard Katz and colleagues at Abbott Laboratories had cloned parts of the erythromycin biosynthetic gene set, using as a probe the resistance gene that Charles Thompson had isolated in his development of cloning vectors (nearly all antibiotic gene clusters contain a self-protective resistance gene as well as the set of biosynthetic genes, and in the early days such easily selected genes were often used to find the more elusive biosynthetic genes). They came to a revolutionary conclusion: the polyketide synthase is a giant protein consisting of "modules" of catalytic sites, or "domains". There were six modules, one for each acyl building block for the carbon chain, so that the programming logic was laid out as an assembly line in which each domain did its job before passing the growing polyketide chain to the next domain. Clearly this situation was ideal for genetic engineering of novel compounds, by mixing and matching modules or domains from different actinomycete gene clusters. Chaitan, with his student Camilla Kao, soon also embraced the erythromycin synthase and there followed a Golden Age of natural product "chemistry by genetics", in academic laboratories and in biotech companies, in which more and more chemists began to exploit the power of molecular biology to practice their trade. There was much focus on the modular polyketides exemplified by the erythromycin paradigm, but soon extending more widely with another major focus on peptide antibiotics, also made on assembly line synthases, but this time putting together chains of amino acids rather than carboxylic acids.
In Part II of my story we have seen how the genetics of Streptomyces and its actinomycete relatives went from the in vivo years to the in vitro era in which sets of genes could be isolated and manipulated with comparative ease. I have focused on my own main interest of antibiotic biosynthesis but the effect of gene cloning in analysing other aspects of actinomycete biology, notably their complex developmental cycle, was equally revolutionary. This was the arena in which Keith Chater, later joined by Mark Buttner and others, including Rich Losick at Harvard, led the charge. In Part III we shall see how sequencing the Streptomyces coelicolor genome at the turn of the millennium opened up new vistas in the understanding of how these organisms are uniquely adapted to their life in the complex soil environment with all its varying chemical, physical and biotic challenges and opportunities.
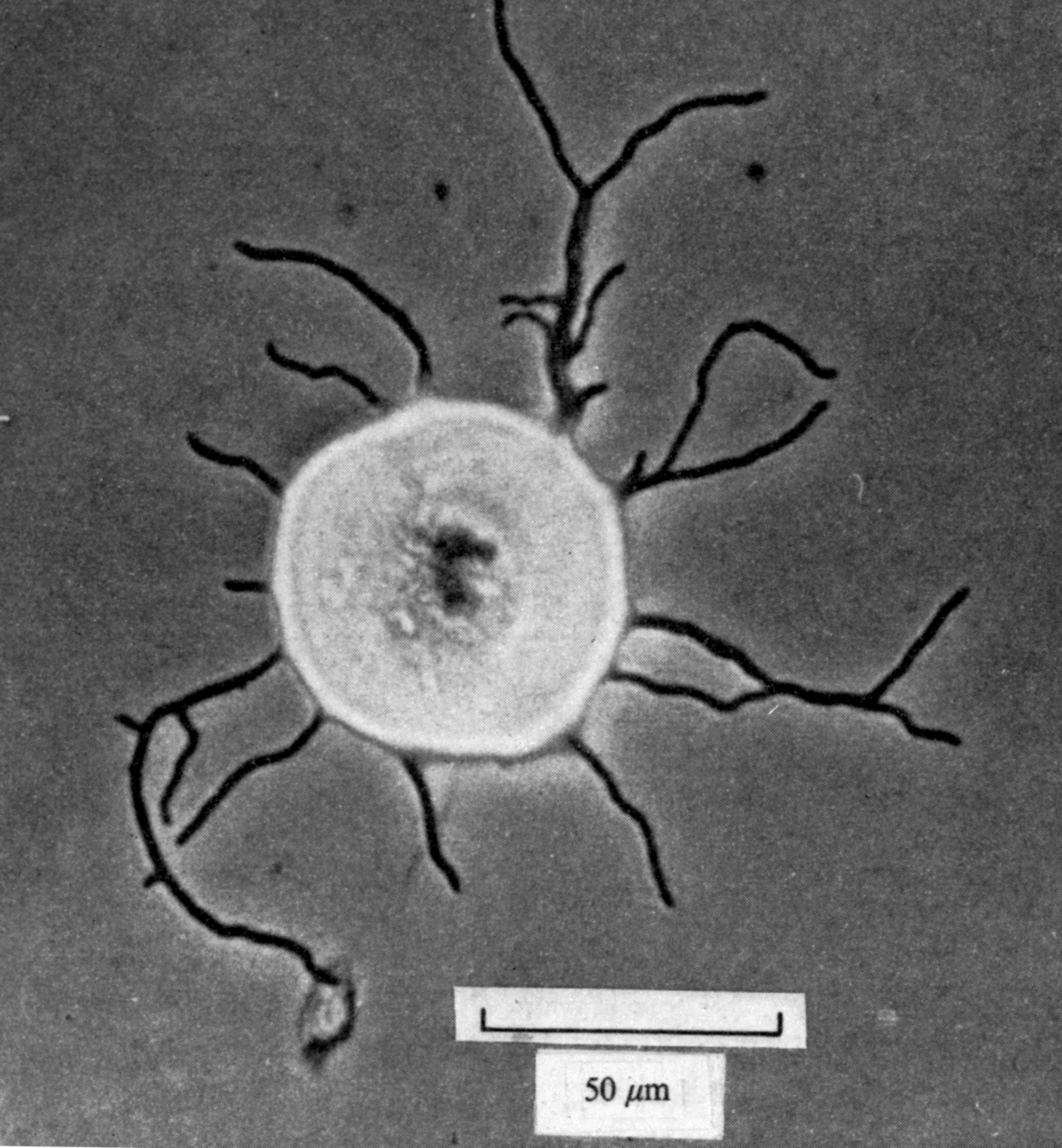
Figure 1. A regenerating Streptomyces protoplast. The protoplast developed into a large sphere, about 20 times the diameter of the original protoplast, and normal-looking hyphae are now growing out from it. (From Okanishi M, Suzuki K and Umezawa H J Gen Microbiol 1974;80:389–400). We proposed that in the initial swelling phase there would be many genomes derived from that of the original protoplast and that if fusion of at least two protoplasts of different genotype had occurred there would be repeated opportunities for recombination before hyphal regeneration, perhaps explaining why recombination via protoplast fusion is so efficient.
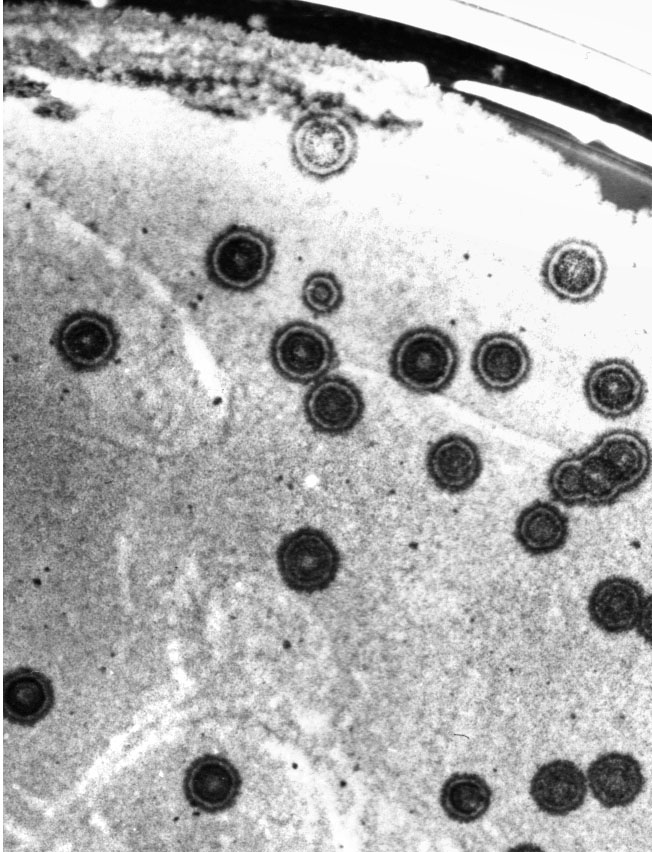
Figure 2. Individual plasmid-containing spores have produced "pocks" on a background of a plasmid-free strain (Courtesy of Tobias Kieser, John Innes Centre).
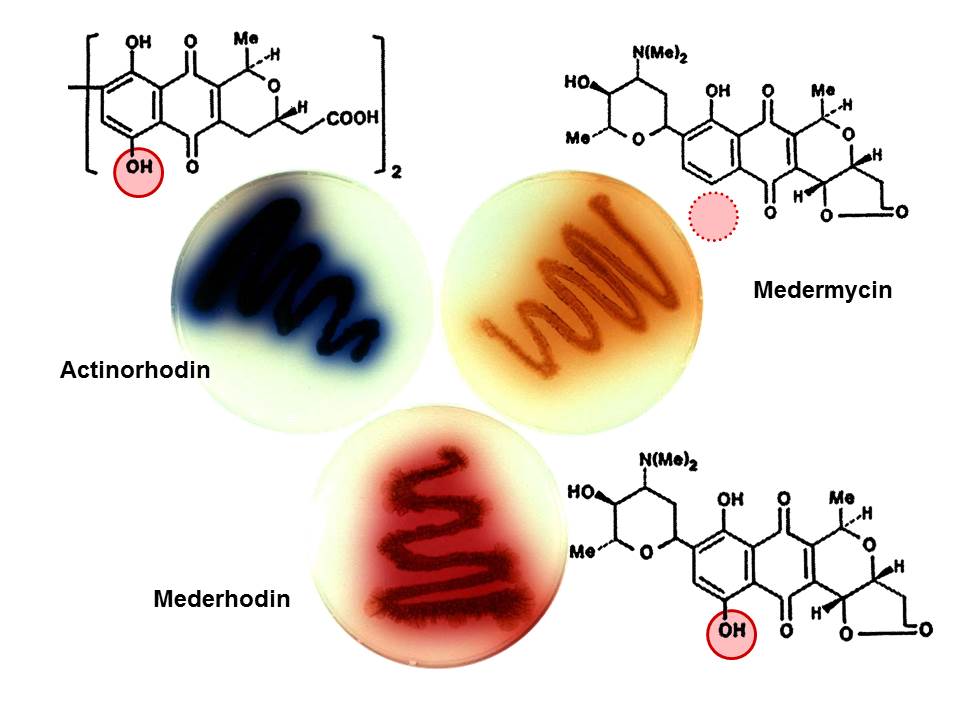
Figure 3. Production of the first "hybrid" antibiotic, the purple mederrhodin, by transfer of genes from the producer of the blue actinorhodin, Streptomyces coelicolor, into the strain making brown medermycin.
Read Part III: The first genome sequence at the turn of the millennium: glimpses of Aladdin’s cave